Abstract
This article shares valuable knowledge and practical know-how on the efficient micellar solubilization of poorly water-soluble active ingredients in microemulsion formulations. These insights can greatly assist in the development of aqueous microemulsion formulas that effectively use these complex A.I.s.
1. Microemulsion and ME pesticide formulation
Microemulsion (ME) formulations are meticulously crafted to produce stable microemulsions when diluted with water, optimizing pesticide efficacy while minimizing environmental and human risks. They enhance the solubility and accessibility of active ingredients, thus improving pest control.
ME formulations are less prone to phase separation and offer extended shelf life while reducing the use of organic solvents, promoting eco-friendliness. These formulations encapsulate solubilized A.I.s within thermodynamically stable microemulsions, spontaneously dispersing upon dilution with water.
The fundamental concept behind ME formulation involves the creation of a microemulsion system that encapsulates solubilized active ingredients (A.I.). Microemulsions are thermodynamically stable systems that exhibit optical transparency and uniformity, formed by the interplay of water and hydrocarbon liquids, held together by a surfactant layer at their interface. Within a microemulsion, you have oil droplets containing surfactant and dissolved, poorly water-soluble A.I. When diluted with water and gently mixed, the microemulsion spontaneously disperses its droplets, essentially undergoing self-emulsification, resulting in the formation of a highly transparent mixture. Such microemulsions maintain their isotropic, thermodynamically stable, and transparent (or partially translucent) characteristics, consisting of oil, water, surfactant, and often a co-surfactant, with droplet sizes falling within the range of 10-100 nm (0.01-0.1 µm). These homogeneous systems, which can be created with varying surfactant concentrations and oil-to-water ratios, all exhibit low viscosity.
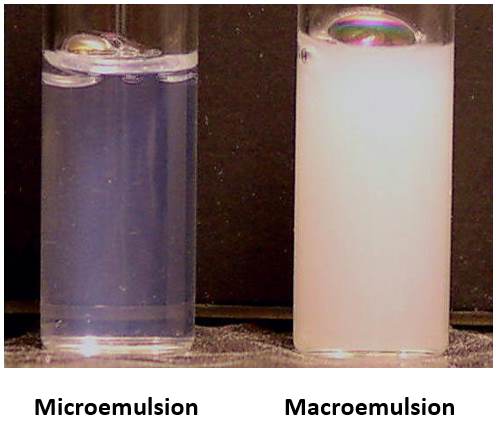
Figure 1. Comparison of appearances of microemulsion and macroemulsion.
Table 1. Difference between microemulsions and macroemulsions
The formation of microemulsions involves several processes, with micellar solubilization playing a pivotal role in their generation. Micellar solubilization serves as a mechanism that enables the incorporation of poorly soluble molecules of active ingredient into nanosized droplets, micelles, effectively "dissolving" or solubilizing a scarcely water-soluble A.I. within the aqueous medium.
To gain a deeper understanding of how the process of micellar solubilization aids in dissolving poorly soluble active ingredients in aqueous formulations, let's delve into this unique phenomenon more closely.
2. Surfactants and Micelles
Surfactants are unique molecules comprising both a hydrophilic portion, known as the "head," and a hydrophobic portion, referred to as the "tail." The head of a surfactant can take various forms: it may carry an electrical charge (either negative, termed "anionic," or positive, known as "cationic"), exhibit a dipolar nature (with both positive and negative charges, referred to as "zwitterionic"), or remain uncharged (referred to as "non-ionic"). Conversely, the tail of a surfactant typically consists of a lengthy hydrocarbon chain.
When surfactants are present in a system at low concentrations, they tend to affix themselves to surfaces or interfaces, leading to a notable alteration in the surface or interfacial energy. Generally, the primary function of surfactants is to diminish this energy. However, when surfactant molecules are dissolved in water at concentrations exceeding a specific threshold known as the critical micelle concentration (CMC), they begin to cluster together, forming entities referred to as micelles.
Within a micelle, the hydrophobic tails aggregate in the central core, distanced from water, while the hydrophilic heads reside on the external surface, in contact with the surrounding water (please refer to Figure 2 for a visual representation). The process of micelle formation in an aqueous environment arises from a delicate equilibrium between various intermolecular forces, encompassing hydrophobic forces, steric forces, electrostatic forces, hydrogen bonding, and Van der Waals interactions. Whether micelle formation takes place and, if so, the concentration of surfactant molecules at which it occurs hinges on the intricate interplay of these forces that facilitate micelle formation.
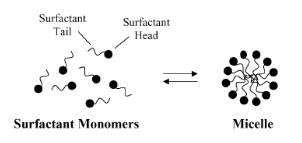
Figure 2: Schematic illustration of the reversible monomer-micelle thermodynamic equilibrium. The black circles represent the surfactant heads (hydrophilic moieties) and the black curved lines represent the surfactant tails (hydrophobic moieties).
Once the surfactant concentration surpasses its critical micelle concentration (CMC), typically falling within the 0.05-0.10% range for most surfactants, micelle formation takes place. During this phenomenon, the A.I.s become ensnared within the micelles. This phenomenon, referred to as micellization, generally leads to an improved solubility of A.I.s that exhibit poor solubility.
KNOW-HOW
To effectively capture poorly soluble A.I. within surfactants and facilitate their solubilization in an aqueous formulation, the formation of micelles is essential. Consequently, it becomes pivotal to maintain a surfactant concentration above the critical micelle concentration (CMC) to ensure stable micelle formation.
The determination of a surfactant's CMC can be accomplished using various physical properties, with the simplest being surface tension. At low concentrations, surfactants tend to align themselves at the liquid's surface, causing only a minimal impact on surface tension. As more surfactant molecules are introduced into the solution, surface tension can rapidly decrease until the liquid's surface is saturated with surfactant molecules, achieving its maximum reduction. At this juncture, the liquid's surface becomes densely packed with surfactant molecules, leading to the self-organization of these molecules into micelles. The Critical Micelle Concentration (CMC) is reached when the surfactant concentration at the surface reaches full saturation, surface tension achieves its maximum reduction, and any additional surfactant molecules must organize themselves into micelles.
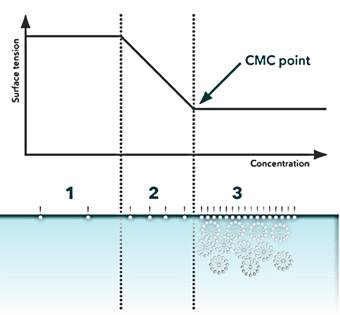
Figure 3. Determination of CMC by assessing surface tension concerning varying surfactant concentrations. This involves plotting surface tension against the logarithm of surfactant concentration.
In case of polymeric surfactants, determination of CMC can be done similarly, however polymeric micelles have CMC in the range which is significantly lower than the CMC of surfactants of low molecular weight, and hence polymeric micelles are thermodynamically more stable even on dilution.
3. Micellar Solubilization
Micelles exhibit an uneven distribution of water within their structure. The concentration of water decreases as one moves from the micelle's surface toward its core, culminating in a completely hydrophobic (water-repelling) core. Consequently, the specific location within a micelle where a solubilized A.I. resides depends on its polarity: nonpolar molecules will be accommodated in the micelle's central core, while substances with intermediate polarity will be dispersed among the surfactant molecules, occupying intermediate positions. These distinct solubilization sites within the micelle arise due to variations in physical properties such as micro viscosity, polarity, and hydration levels that are not consistent throughout the micelle's structure. Figure 4 illustrates potential sites for the solubilization of an A.I. within a micelle.
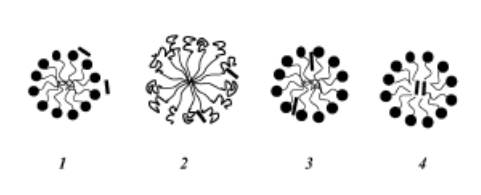
Figure 4. Solubilization sites within a surfactant micelle. The A.I. molecule can be found at different sites within the micelle, as denoted by the black bold lines (⎯). The surfactant heads are represented by black circles, while the black bold curved lines depict the surfactant heads, and the light black curved lines represent the surfactant tails.
The ability of surfactants to dissolve A.I.s depends on a multitude of factors, including the chemical makeup of the surfactant, the chemical composition of the A.I. molecule, temperature, pH levels, ionic strength, and more. Typically, water can solubilize poorly water-soluble A.I. to a degree of up to 1%. When dealing with hydrophobic A.I. molecules, non-ionic surfactants are often more effective solubilizing agents than ionic surfactants due to their lower critical micelle concentration (CMC) values.
For polar A.I.s, establishing a general relationship between the degree of solubilization and the chemical structure of the surfactant is more intricate, as solubilization can occur in both the inner and outer regions of the micelle. Generally, if the A.I. molecule is less polar (or has weaker interactions with the polar head of the surfactant within the micelle or with water molecules at the micelle-water interface) and possesses a longer chain length, it tends to exhibit a lower degree of solubilization, indicating deeper penetration into the palisade layer. The extent of solubilization within a specific micelle is contingent upon the solubilization site and, consequently, the micelle's shape.
Regarding the impact of the A.I.'s structure, crystalline solids typically exhibit lower solubility within micelles compared to liquids with a similar composition. As a practical suggestion, it is recommended to first dissolve solid A.I. in a suitable solvent and subsequently endeavour to solubilize the organic phase containing the dissolved A.I. in water.
As a general trend, the quantity of A.I. that can be solubilized in a micellar system tends to increase as the temperature rises. This phenomenon can be attributed to the heightened thermal agitation, resulting in greater space availability for solubilization within the micelle. Additionally, higher temperatures often lead to increased solubility in water. For non-ionic surfactants like polyoxyethylene (POE), the impact of temperature on the extent of A.I. solubilization may be contingent on whether the A.I. molecule is situated inside the hydrophobic core or within the palisade layer.
Ionic strength can exert a significant influence on the solubilization of an A.I. in micellar solutions, especially when dealing with ionic surfactants. The introduction of even small quantities of salts serves to reduce the repulsion between the similarly charged ionic surfactant head groups, consequently lowering the critical micelle concentration (CMC) and enhancing the aggregation and volume of the micelles. This increased aggregation promotes the solubilization of hydrophobic A.I. molecules within the inner core of the micelle. Moreover, the addition of salts to solutions containing PEO non-ionic surfactants may also amplify the degree of solubilization of hydrophobic molecules.
Polymeric micelles are constructed from copolymers containing both hydrophilic and hydrophobic monomer units, such as PEO (polyethylene oxide) and PPO (polypropylene oxide), respectively. These amphiphilic block copolymers, with the hydrophilic block typically surpassing the length of the hydrophobic block, can arrange themselves into spherical micelles when placed in aqueous solutions. Within these micelles, the core comprises the hydrophobic blocks, while the outer region consists of the hydrophilic blocks. For a visual representation of the process of polymeric micelle formation, please refer to Figure 5.
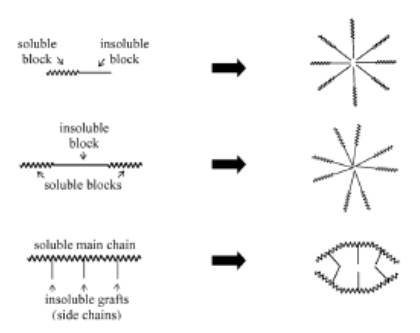
Figure 5. Formation of polymeric micelles from different types of amphiphilic block co-polymers
Most of the research and practical applications conducted thus far have centred around block copolymers composed of PEO and PPO blocks (block copolymers in which central polypropylene glycol group is flanked by two polyethylene glycol groups), which are commercially recognized as Pluronics®. Pluronic PE product line of BASF varies by molar mass of polypropylene glycol block and percentage of polyethylene glycol in molecule. See also the article on https://michberk.com/polymericdisperants.aspx.
KNOW-HOW
An enhancement in the aqueous solubility can be achieved through the addition of hydrotropic agents. Hydrotropism represents a type of solubilization process where the introduction of a substantial quantity of a hydrotropic agent, typically a water-soluble organic salt, results in an increase in the aqueous solubility of a poorly soluble A.I. Hydrotropic agents are essentially ionic organic salts, composed of alkali metal salts derived from various organic acids.
Additives or salts that augment solubility in a solvent are described as "salting in" the A.I., whereas those that reduce solubility are said to "salt out" the A.I. Certain salts with large anions or cations that themselves have high solubility in water induce the "salting in" effect for non-electrolytes. These salts are termed "hydrotropic agents," and the phenomenon is referred to as "hydrotropism." Hydrotropism denotes the increase in solubility in water due to the presence of a significant amount of additives. The mechanism behind its ability to enhance solubility is closely tied to complexation, involving a weak interaction between hydrotropic agents such as sodium benzoate, sodium acetate, sodium alginate, urea, and poorly soluble A.I.s.
Hydrotropes are known to self-assemble in solution. Examples may encompass ethanol, aromatic alcohols, and ionic surfactants like acids such as SDS (sodium dodecyl sulfate) and sodium dodecylated oxidibenzene disulfonate. Aromatic hydrotropes with anionic head groups have been the focus of extensive research. Cationic hydrotropes with hydrophilic groups are less common, represented by salts of aromatic amines, for instance.
KNOW-HOW
Additional types of emulsifiers that can prove beneficial for ME formulations pertain to the following categories of surfactants.
Sorbitan esters
Structure |
Application |
Sorbitan laureates
Sorbitans palmitate
Sorbitans stearate
Sorbitan tristearate
Sorbitan isostearate
Sorbitan oleate
Sorbitan sesquioleate
Sorbitan trioleate
|
Fatty acid and Sorbitan esters. Non-ionic emulsifiers from vegetable origin with a lipophilic tendency (HLB between2-8) |
Polysorbates
Structure |
Application |
Polysorbate 20
Polysorbate 40
Polysorbate 60
Polysorbate 80
Polysorbate 81
Polysorbate 85
|
Sorbitan ester ethoxylated derivatives. Non-ionic emulsifiers from vegetable origin with a hydrophilic tendency (HLB between 10-17). All grades are guaranteed high purity (DF=1-4 Dioxane free) |
POE fatty acids
Structure |
Application |
PEG – 8 Stearate
PEG – 40 Stearate
|
Polyethoxylated fatty acids. Non – ionic emulsifiers from vegetable origin with a hydrophilic tendency (HLB between 11 – 19) |
POE fatty Alcohols
Structure |
Application |
Ceteareth-33
Laureth-23
Ceteth-20
Ceteareth-20
Oleth-20
|
Polyethoxylated fatty alcohols. Non-ionic emulsifiers with a hydrophilic or lipophilic tendency depending on the HLB |
Self-emulsifying bases
Structure |
Application |
Cetearyl alcohol & Cetereth 33
PEG 100 Stearate & Glyceral stearate
|
Non-ionic self-emulsifying bases for formulation of oil/water emulsions with various consistencies. |
W/O emulsifiers
Structure |
Application |
PEG -30 dipolyhydroxystearate
|
Polymeric emulsifier effective at low dosage to obtain perfectly stable water-in-oil emulsions with high water content. |
4. Techniques of ME formulation
KNOW-HOW
Below, you'll find a few illustrations of solubilization systems suitable for ME formulations.
Example 1
Liquid A.I.s (which are oily) or solid A.I.s dissolved in an organic solvent are rendered soluble in a surfactant/water system, instead of relying on the organic solvent. Different ratios of surfactant to water, specifically 1:1, 2:1, and 3:1, are employed for this purpose. The surfactants of choice are typically TWEEN 20 and TWEEN 80, both of which are non-ionic polysorbates. TWEEN 20 is frequently the preferred option for solubilizing substantial quantities of oily A.I.s.
To ascertain the optimal surfactant-to-water ratio, a straightforward experiment can be conducted. In this experiment, an oily A.I. is added drop by drop into vials containing the various surfactant-to-water ratios, with thorough mixing (vortexing) after each addition. The titration continues until the entire solution remains clear and transparent. The endpoint of the titration is determined by the first drop of oily A.I. that causes the surfactant/water solution to become cloudy without returning to a transparent state. All samples within the ternary system comprising surfactant/water/A.I. are then left at room temperature for an additional hour and subsequently examined visually for transparency.
Example 2
Solubilizers:
TWEEN 80 (polysorbate 80) is miscible in water yielding a clear to slightly hazy faint yellow solution. It is reported to be miscible with alcohol, cottonseed oil, corn oil, ethyl acetate, methanol, and toluene, but insoluble in mineral oil
TWEEN 20 (polysorbate 20) is soluble in water, alcohols (ethyl, methyl, isopropyl), ethylene and propylene glycol and is insoluble in mineral oils)
Water
Preservative (e.g., phenoxyethanol)
pH adjuster (if necessary)
Procedure:
Weigh Ingredients: Begin by weighing the A.I. and the solubilizer. The solubilizer is crucial for solubilizing the oil based A.I. in water
Mix Solubilizer and A.I. in the appropriate ratio. The specific ratio may vary depending on the solubilizer and A.I. used. Conduct a compatibility test to determine the right ratio
Stir Gently: Stir the mixture gently to ensure thorough blending. This initial mixture may appear cloudy
Add Water: Gradually add water to the mixture while stirring continuously. The solubilizer should help disperse the oily A.I. evenly in the water
Continue Stirring: Continue stirring until the mixture becomes clear or translucent. This indicates that the A.I. has been solubilized in the water
pH Adjustment: If necessary, adjust the pH of the solution to the desired range for your formulation product. Most solubilizers work well in a slightly acidic to neutral pH range
Preservative Addition: To ensure the stability and safety of the formulation, add a suitable preservative (e.g., phenoxyethanol) according to the recommended dosage. Mix it thoroughly
Example 3, 4, 5
Solubilizing system |
% weight |
Active Ingredient |
1 |
1 |
1 |
TWEEN 20 |
10 |
- |
5 |
TWEEN 60 |
- |
12 |
3 |
WATER |
89 |
87 |
91 |
Example 6
Solubilizing system |
% weight |
Active Ingredient |
1 |
1 |
1 |
TWEEN 80 (Polysorbate 80) |
15 |
- |
6.3 |
TWEEN 85 (Polysorbate 85) |
- |
15 |
3.7 |
WATER |
84 |
84 |
89 |
4. Conclusion
This article emphasizes the critical role of micellar solubilization in enhancing the solubility of poorly water-soluble active ingredients within microemulsions. It presents a range of Know-How to achieve successful solubilization, including determining CMC, emphasizing the importance of maintaining a concentration above this critical point. The article then delves into approaches for enhancing aqueous solubility by incorporating hydrotropic agents and selecting emulsifiers. The provided examples can serve as a roadmap for developing efficient pesticide formulations using microemulsions.